By Dan Rubinstein
Photos by Luther Caverly and Chris Roussakis
David Sinclair stands at the bottom of a cylindrical cavern two kilometres beneath the rolling scrubland of Sudbury, Ont. The walls of the Cryopit at SNOLAB — a world-class science laboratory accessed via a spur tunnel from an active nickel mine — are shiny white trowel-smooth shotcrete. The concrete floor is covered with an epoxy finish to seal in surface dust and create a washable surface. But other than some blue ventilation tubes hooked up to the facility’s air purification system, a few bundles of cable and a couple pallets of shrink-wrapped electronics equipment being stored here temporarily, the enormous space — 20 metres high, with a diameter of 15 metres — is empty for now.
That doesn’t deter Sinclair, an internationally renowned particle physicist and a distinguished research professor at Carleton University. He’s focused on what the Cryopit could soon contain: one of the proposed uses for the space is the cutting-edge nEXO experiment, a detector that will use five tonnes of enriched xenon in an attempt to measure the exact mass of neutrinos and determine whether the elusive elementary particles are their own antiparticles. Sinclair is also thinking about the past.
He’s reminiscing about the mid-1980s, when he was a young faculty member at the University of Oxford — before the Sudbury Neutrino Observatory (SNO) experiment was built and helped a Canadian win the Nobel Prize in Physics, before the federal government decided to double down on its initial investment and fund the construction of SNOLAB, before his Carleton colleague Mark Boulay began the DEAP-3600 project in the adjacent cavern to search for an unobserved form of matter that comprises most of the mass in the universe — and all of this was solid rock.
Although he is always careful to share credit and stresses that it takes a lot of people to make a project of this scale feasible, Sinclair made a crucial decision while at Oxford that ultimately begat the SNO experiment and the thriving research legacy that followed. There had been talk in Canada’s particle physics community about the possibility of an underground lab in the Sudbury area, with hundreds of metres of norite shielding sensitive detection systems from the cosmic radiation that bombards the planet. Sinclair was excited about the idea — and the opportunity to probe fundamental questions about the nature of the universe on home turf — but knew it would never proceed if people only discussed it over coffee and chipped away at the concept while concentrating on their day jobs. He had a sabbatical leave coming up in 1984-‘85 and planned to spend it working in Australia, an appealing adventure for an ex-pat Canadian and his family.
“I was free to go anywhere in the world and do anything that I wanted,” recalls Sinclair, looking up at the domed ceiling of the Cryopit. “But what the SNO project needed was somebody with a year of free time to take on the feasibility study. So I called up my wife and said: ‘We’re not going to Australia after all — we’re going back to Canada.’ And I’ve been working on this ever since.”
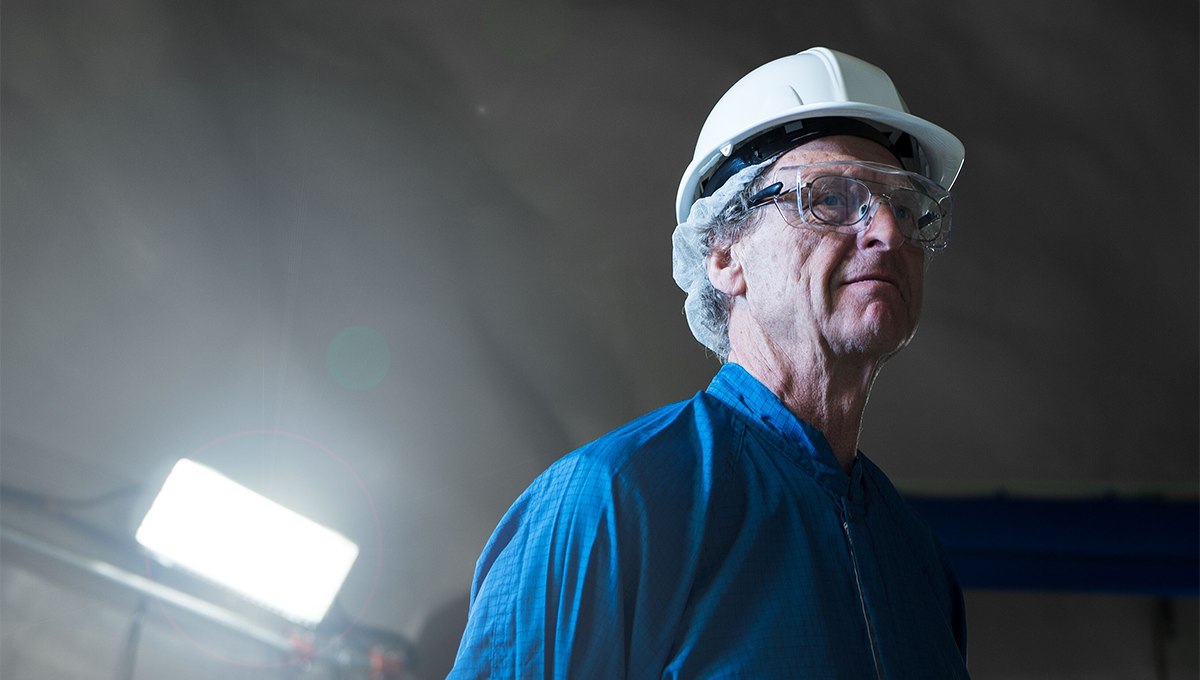
The Hunt for the Neutrino Begins
Funded by a collection of agencies, including Canada’s Natural Sciences and Engineering Research Council (NSERC) and National Research Council, Industry Canada, the Northern Ontario Heritage Fund Corporation, the U.S. Department of Energy and the U.K. Particle Physics and Astronomy Research Council, the SNO Institute was formed in 1990. About 100 scientists from Canada, the U.S. and the U.K. comprised the team; its director was Queen’s physicist Art McDonald — who would go on to win the 2015 Nobel Prize — and Sinclair served as one of his deputies. Excavation began in March 1990 and was completed just over three years later.
Sinclair — who was born in Montreal and grew up in Ottawa before doing a BSc and PhD in physics at Queen’s and then a postdoc at the Niels Bohr Institute in Copenhagen — lived in Sudbury with his family during construction and commissioning of the SNO experiment. An avid fisherman who frequently goes casting in Quebec and northern Canada, he brought a rod and reel and occasionally dropped a line into local lakes and nearby Georgian Bay. But the real prize he was seeking was not so simple.
The neutrino was first postulated by theoretical physicist Wolfgang Pauli in 1930 to explain why energy did not appear to be conserved in certain types of radioactive decay, which is defined as “the spontaneous transformation of an unstable atomic nucleus into a lighter one.” The missing energy, Pauli suggested, was carried off by a tiny subatomic particle with zero electrical charge (neutrino is Italian for “little neutral one”). Thought to be among the most abundant particles in the universe, they were exceedingly difficult to detect. “About 100 billion neutrinos from the sun pass through your thumbnail every second,” according to the Nobel Prize website, “but you do not feel them because they interact so rarely and so weakly with matter.”
The Sudbury Neutrino Observatory consisted of 1,000 tonnes of heavy water (on loan from Atomic Energy of Canada Ltd.) inside a spherical acrylic vessel with a 12-metre diameter. This vessel was immersed in ultra pure water in a barrel-shaped cavern 2,070 metres below the Earth’s surface; 30 metres tall, with a diameter of 22 metres, it’s the largest cavern at this depth in the world. SNO was operated as a cleanroom lab: scientists, technicians and visitors had to shower and put on clean coveralls, shoes and hairnets before entering the facility, eliminating the trace levels of radiation that are emitted from the everyday dirt on our clothing, skin and hair.
The 10 or so neutrinos that interacted with the detector every day would be stopped or scattered by the heavy water, Sinclair and his colleagues hypothesized, producing flashes of light called Cherenkov radiation. This light would be detected by an array of 9,600 photomultiplier tubes (PMTs) mounted on a geodesic support structure surrounding the heavy water vessel. The flashes would be recorded and analyzed, allowing scientists to extract information about the neutrinos that caused them. The lab included electronics and computer facilities, a control room, and water purification systems for both heavy and regular water. A trailer near the mine shaft on the surface would function as its office.
As often occurs with experiments of this scale and complexity, there was a hiccup when the SNO detector was turned on for the first time in 1999. PMTs are a widely used technology in particle physics experiments but are not normally submerged in water. The PMTs for SNO were water tested at Carleton and seemed to work fine, but in the detector, running at about 2,000 volts, they started to spark. “It was a dark day for the SNO experiment,” recalls Sinclair, who was approached by McDonald and asked to find a solution. Sinclair had designed SNO’s innovative water purification system — the experiment required water with about a million times less radioactivity than drinking water — and had the technical experience (and engineering and chemistry knowledge) to address the problem. Eventually, he realized that because the PMTs were immersed in water from which all the standard dissolved gasses had been extracted, the gas-permeable seal in the PMTs allowed gas in the tubes to diffuse into the water. This was creating a vacuum at the base of the PMTs, and as the pressure dropped, the voltage capability also dropped. Sinclair came up with a way to introduce very clean nitrogen into the water, and the sparking stopped.
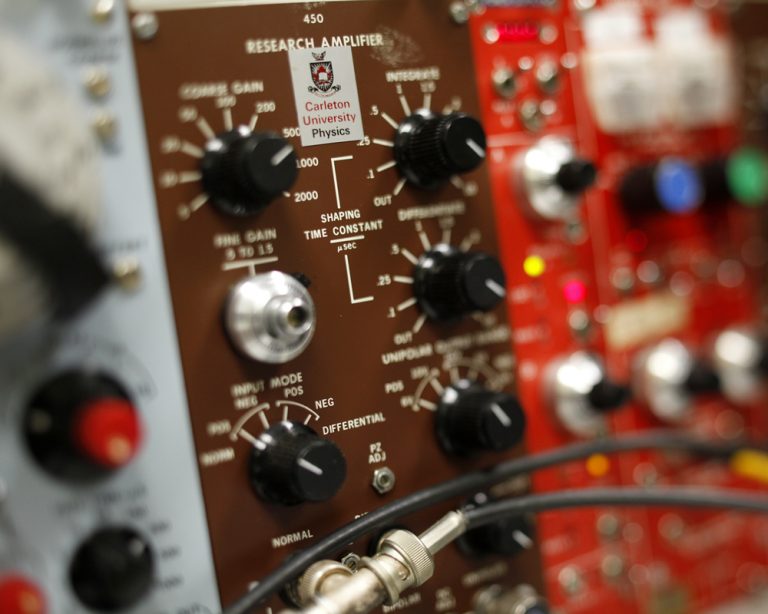
Solving the Neutrino Mystery
With the detector functioning properly, data collection and analysis could begin. In 2001, the SNO team released its first results — and, right out of the gate, solved a 30-year-old mystery.
Since the early 1970s, scientists have known that electron-neutrinos — one of three types of the particle, along with the muon-neutrino and the tau-neutrino — are emitted in vast numbers by the nuclear reactions that fuel the sun. But experiments that detected neutrinos reaching Earth “found only a fraction of the number expected from detailed theories of energy production in the sun. This meant there was something wrong with either the theories of the sun, or the understanding of neutrinos.” SNO researchers discovered that some of the electron-neutrinos change into other types of neutrinos as they travel to the Earth.
“The determination that the electron neutrinos from the sun transform into neutrinos of another type is very important for a full understanding of the universe at the most microscopic level,” SNO scientists said in a news release. “This transformation of neutrino types is not allowed in the Standard Model of elementary particles. Theoreticians will be seeking the best way to incorporate this new information about neutrinos into more comprehensive theories. The direct evidence for solar neutrino transformation also indicates that neutrinos have mass.”
That finding meshed with the results from the Super-Kamiokande detector in Japan, earning McDonald and Takaaki Kajita the Nobel Prize. “The discovery has changed our understanding of the innermost workings of matter and can prove crucial to our view of the universe,” the Royal Swedish Academy of Sciences said in a release. “Now the experiments continue and intense activity is underway worldwide in order to capture neutrinos and examine their properties. New discoveries about their deepest secrets are expected to change our current understanding of the history, structure and future fate of the universe.”
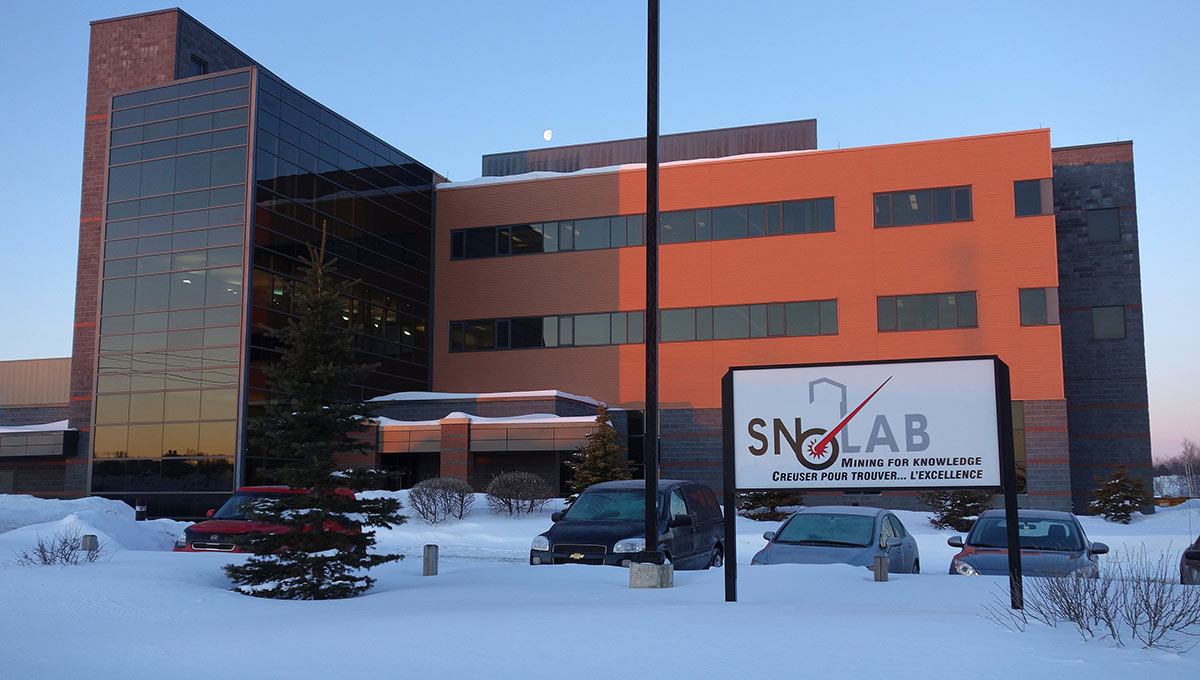
Descending into the Western Hemisphere’s Deepest Mine
To visit SNOLAB, a half-hour drive west of downtown Sudbury, you must arrive early in the morning at the facility’s surface building, a 3,000-square-metre, three-storey complex completed in 2005 to replace the original office trailer. In the gear room, you’re given heavy-duty neon yellow coveralls, boots, a hardhat, headlamp and safety glasses and, after changing, you walk a couple hundred metres to the No. 9 shaft — the main access portal to Vale’s Creighton Mine and, at 2,175 metres, the deepest continuous mine shaft in the Western Hemisphere.
Scientists and guests squeeze into a double-decker elevator (known as a cage) with a few dozen miners beginning their shift, the gate is closed, and you descend at up to 50 kilometres an hour to 2,070 metres below ground. You step out of the cage into a dark tunnel (or drift) carved out of the rock, with walls covered in metal screening, and walk about a kilometre and a half, stepping aside to let mine vehicles pass, until reaching the entrance to the lab. Then you shower, put on clean clothing and enter a bustling 5,000-square-metre warren of scientists at work.
SNOLAB has space for about 120 people per shift. The cavern that contained SNO — which stopped taking data in 2006 — is now home to the SNO+ experiment, which repurposed the original detector (replacing the heavy water with liquid scintillator, an organic liquid similar to mineral oil that gives off light when charged particles pass through it) for a study of low-energy solar neutrinos and other physics phenomena. There’s also a new Cube Hall cavern that’s ground zero for Carleton researcher Mark Boulay’s DEAP experiment. The Cryopit is SNOLAB’s other large space, but the cleanroom facility also includes smaller “ladder labs” in the drifts that connect the caverns, a lunchroom and meeting room.
There was no guarantee that any of this would be built. SNO was intended to be a single experiment, not a facility, says Sinclair, because the latter implied a long-term commitment that funders were not prepared to make. “But SNO was such a success that we changed that idea,” he says. “Making a facility for a series of experiments was seen as the right thing to do. Doing a single experiment has two problems. First of all, these experiments take a lot of planning and design, so there’s a long period where you’re testing ideas. For SNO, that was over a decade, and it took about a decade to build the experiment, and a couple more years before we had the answers we were looking for. But if you want a facility that attracts students and young scientists, having a sequence of experiments so there’s always something that’s coming up with exciting results — and something that’s big enough so there are always different groups coming together — that’s a wonderful fertile environment for students and young scientists.”
It cost $70 million to build SNOLAB, with excavation taking place in 2007 and 2008, and cleanroom status achieved in 2010. The Cryopit — the third-largest space after the SNO cavern and Cube Hall — was designed specifically for cryogenic detectors; it’s isolated within the facility in case the detector warms and water needs to be vaporized and expelled.
Darryl Boyce, Carleton’s assistant vice-president of Facilities Management and Planning, played a critical role in the construction of SNOLAB. “He provided outstanding management of the project,” says Sinclair, “just as if it were being carried out on the Carleton campus.”
More than half the total funding was provided by the Canada Foundation for Innovation (CFI). The remainder came from the Ontario Innovation Trust, the Northern Ontario Heritage Fund and FedNor, with the CFI, NSERC and member institutions supplying operating funding, and the City of Greater Sudbury supporting public education. In January 2017, the CFI provided $28.6 million for three years of operations, and six months later the Ontario government added a $28.8- million five-year boost.
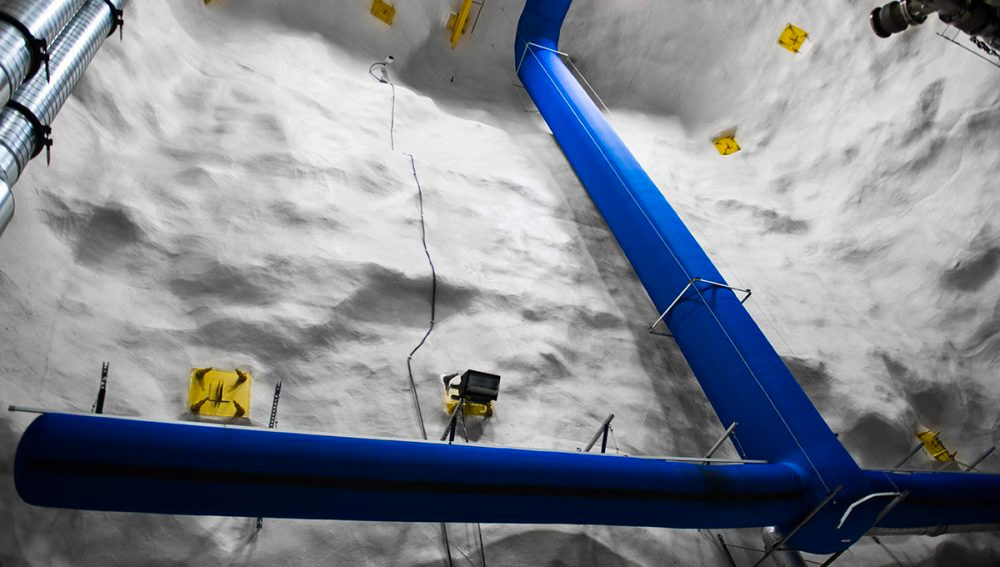
Focusing on Neutrino Mass with the nEXO Experiment
Even though he is officially retired — a move he made three years ago to allow Carleton to hire new physics faculty — Sinclair is in line at a campus Tim Hortons at 8 a.m. after a late-night flight back to Ottawa from Sudbury. He’s still working full time, going to his lab every day and supervising grad students, with the nEXO experiment occupying most of his research attention. Sinclair has been involved with the project since it started a dozen years ago, when EXO-200 — a prototype detector using 200 kilograms of liquid argon inside a vessel made from thin, ultra-pure copper — was built at Stanford. In 2007, the detector was moved to the Waste Isolation Pilot Plant, a geological repository for nuclear waste 650 metres below ground near Carlsbad, New Mexico.
The detector is looking for evidence of a process known as neutrinoless double beta decay, a type of radioactive decay in which two protons are simultaneously transformed into two neutrons, or vice versa, inside an atomic nucleus. Observing any signs of this process, says the Institute of Physics magazine Physics World, would show that neutrinos are their own antiparticles (a particle with the same mass but opposite charge). “This would constitute discovering a new class of particles that lies beyond the Standard Model of particle physics,” says Physics World, “and would be a major breakthrough in modern physics.” Measurements of this decay process could also be used to determine the absolute mass of a neutrino.
Sinclair, who chairs the international nEXO collaboration’s board and is its spokesperson in Canada, is concentrating on the conceptual design for the full-scale detector that could be installed in SNOLAB’s Cryopit. The push for funding — “at the couple hundred million dollar level,” he says — is under way. The project, which involves scientists from the United States, Russia, China and Germany, is looking for financial support from U.S. Dept. of Energy, among other potential backers interested in this type of physics. The experiment is so expensive in part because it will need five tonnes of isotopically enriched xenon, which alone is worth about $100 million. To get that amount, 50 tonnes of normal xenon must be put through an isotope separator — and global production of xenon, a byproduct of liquid oxygen used in the steel industry, is only about 40 tonnes a year, much of it ending up in car headlights, plasma televisions and spacecraft ion drives.
If the funding for nEXO was secured tomorrow, it would still take about six years to build the experiment and another six years to take data. But to Sinclair, the wait — and effort — is worth it. “People have been looking for double beta decay for decades, and the discovery that neutrinos have mass means that we now know where to look,” he says. “It’s a very challenging experiment still, but we know it’s not infinitely challenging, so it has new scientific impetus.
“We set up this lab to do experiments that are absolutely fundamental to our understanding of the universe,” he continues. “It’s a very competitive and challenging field, to come up with experiments that can distinguish the very feeble signals we’re looking for from the backgrounds that normally surround us with radioactivity and cosmic rays and all kinds of other surface noise that we’ve avoided by coming deep underground.
“In addition to the scientific legacy we’re building on, there’s a technical one. All the lessons we learned in making a deep underground experiment, in making very pure materials and keeping the whole system very clean, not only did we learn how to do this, but we also convinced the world that it’s possible to do.”
In the echo of the empty Cryopit, Sinclair explains the significance of nEXO, which can trace its lineage directly to SNO. The breakthrough Higgs boson, which gives mass to all particles, might not work for neutrinos, which are much lighter than any other fundamental particle. It appears that a different mechanism may provide mass to the neutrino. If the neutrino is its own antiparticle, that theory makes sense, and double beta decay can only occur if the neutrino is its own antiparticle. “The physics would allow a different way to provide mass to such a particle,” says Sinclair. “It would go a long way toward understanding the structure of the fundamental particles of the universe.
“But there’s another motivation, a cosmological mystery that we’ve been struggling with for a long time,” he adds. “Why is the universe here? Or, at least, why are we in it? We understand the big bang, that the universe started in a state of enormous energy. This energy can produce particles and antiparticles, and particles and antiparticles can annihilate back into energy, and this happened over and over and over again as the universe cooled and expanded. But at the end of that process, we were left with just particles. So there must be a symmetry between matter and antimatter, particles and their antiparticles, and we don’t know what that is.
“One of the really surprising outcomes of SNO — maybe the most significant outcome of SNO — is that maybe the answer lies in the properties of neutrinos. The arguments are somewhat indirect, but one of the criteria is that we have to understand the question as to whether neutrinos are their own antiparticles. Other aspects of the theory are going to be very challenging to test, but this is one of the windows that we can look at and see if we’re on the right track to understanding this very important fundamental property of the universe — that we’re here. Because if we didn’t have the asymmetry, by the time the annihilation was over, we would be left with a scattering of particles and antiparticles at such low density that they never interacted. So, we’d never get matter. We’d never get material forming. We’d never form. It’s absolutely critical to understanding the universe — that, to me, is the main reason we’re here.”
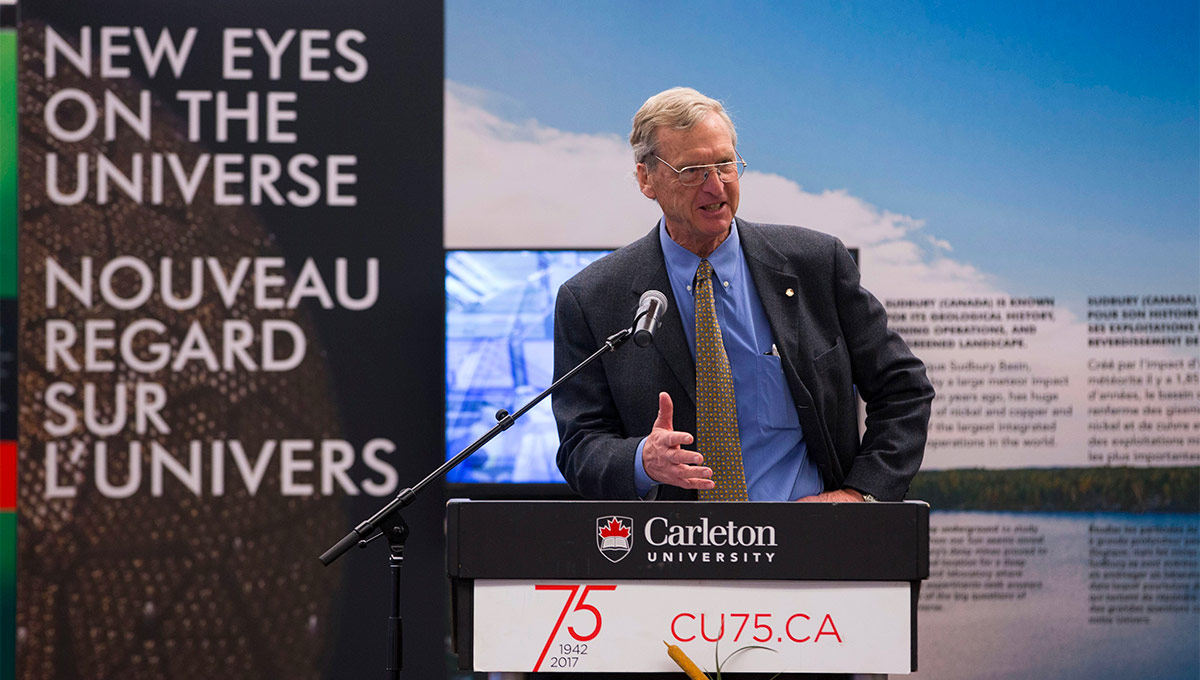
Monday, October 16, 2017 in Faculty of Science, Research
Share: Twitter, Facebook